Bits of the stars are all around us, and in us, too. About half of the abundance of elements heavier than iron originates in some of the most violent explosions in the cosmos. As the universe churns and new stars and planets form out of old gas and dust, these elements eventually make their way to Earth and other worlds. After 3.7 billion years of evolution on our planet, humans and many other species have come to rely on them in our bodies and our lives. Iodine, for instance, is a component of hormones we need to control our brain development and regulate our metabolism. Ocean microplankton called Acantharea use the element strontium to create intricate mineral skeletons. Gallium is critical for the chips in our smartphones and our laptop screens. And the mirrors of the JWST are gilded with gold, an element useful for its unreactive nature and ability to reflect infrared light (not to mention its popularity in jewelry).
Scientists have long had a basic idea of how these elements come to be, but for many years the details were hazy and fiercely debated. That changed recently when astronomers observed, for the first time, heavy-element synthesis in action. The process, the evidence suggests, went something like this.
Eons ago a star more than 10 times as massive as our sun died in a spectacular explosion, giving birth to one of the strangest objects in the universe: a neutron star. This newborn star was a remnant of the stellar core compressed to extreme densities where matter can take forms we do not understand. The neutron star might have cooled forever in the depths of space, and that would have been the end of its story. But most massive stars live in binary systems with a twin, and the same fate that befell our first star eventually came for its partner, leaving two neutron stars circling each other. In a dance that went on for millennia, the stars spiraled in, slowly at first and then rapidly. As they drew closer together, tidal forces began to rip them apart, flinging neutron-rich matter into space at velocities approaching one-third the speed of light. At last the stars merged, sending ripples through spacetime and setting off cosmic fireworks across the entire electromagnetic spectrum.
At the time of the crash, our own pale blue planet, in a quiet part of the Milky Way about 130 million light-years away, was home to the dinosaurs. The ripples in spacetime, called gravitational waves, began making their way across the cosmos, and in the time it took them to cover the vast distance to Earth, life on the planet changed beyond recognition. New species evolved and went extinct, civilizations rose and fell, and curious humans began looking up at the sky, developing instruments that could do incredible things such as measure minute distortions in spacetime. Eventually the gravitational waves (traveling at light speed) and the light from the merger reached Earth together. Astrophysicists recognized a distinctive glow that showed the presence of new elements. Humanity had just witnessed heavy-element production.
As an expert in cosmic cataclysms, I’m enthralled by both the science and the romance of this story—the creation of something new and enduring, even precious, from an ancient remnant of a once luminous star. And I’m thrilled that we finally get to see it happening. The discovery has answered several long-standing questions in astrophysics while also raising entirely new questions. But I and many scientists are energized. Our newfound ability to detect gravitational waves, as well as light from the same cosmic source, promises to help us understand astrophysical explosions and the synthesis of elements in a way that was previously impossible.
We Are Stardust
The quest to understand heavy-element formation is part of a larger scientific effort to answer a fundamental question: Where did everything come from? The cosmic history of the elements of the periodic table extends from a few minutes after the big bang to the present. The synthesis of the first elements—hydrogen, helium and lithium—occurred roughly three minutes after the birth of the universe. From these ingredients, the first stars formed, shining bright and fusing new elements in their cores during both their lives and their explosive deaths. The next generation of stars was born from the debris of these blasts, enriched with the elements formed by the first stars. This process continues today and accounts for all the elements from helium on the light end, with two protons per atom, all the way up to iron, which has 26 protons in its atomic nucleus. The heaviest elements, such as tennessine with 117 protons, aren’t created by nature at all. But physicists can force them into being inside particle accelerators, where they typically last for mere thousandths of a second before decaying.
Several decades ago scientists theorized that about half of the elements heavier than iron are produced through a process called rapid neutron capture, or the r-process. The rest are thought to originate through slow neutron capture, or the s-process—a relatively well-understood sequence of reactions that occurs in long-lived, low-mass stars.
Both the r-process and the s-process involve adding one or more neutrons to an atomic nucleus. Adding neutrons, however, does not produce a new element, because elements are defined by the number of protons in their nucleus. What we do get is a heavier isotope of the same element—a nucleus containing the same number of protons but a different number of neutrons. This heavy isotope is often unstable and radioactive. Through what’s called beta-minus decay, a neutron will transform into a proton, spitting out an electron and another subatomic particle called a neutrino in the process. In this way, the number of protons in an atom’s nucleus increases, and a new element is born.
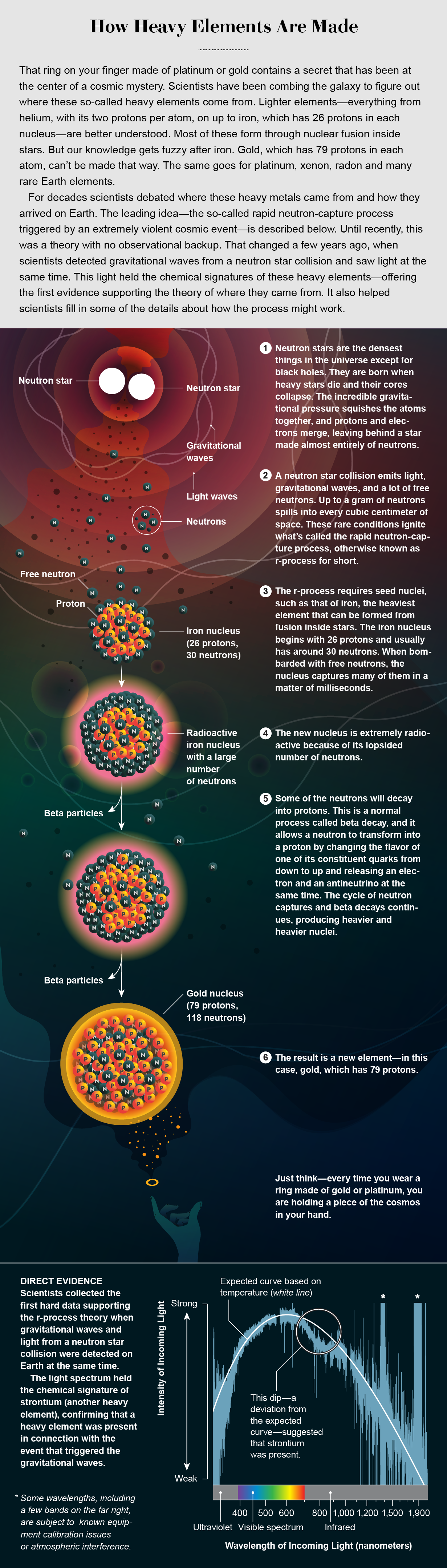
The key difference between the s-process and the r-process is speed. In the s-process, atoms capture neutrons slowly, and there is plenty of time for the newly added neutron to decay into a proton, creating the next stable element in the periodic table—with just one proton more—before another neutron comes along to be captured. This happens over thousands of years because there are only small numbers of extra neutrons lying around in the stars that host the s-process, so atoms are able to capture new neutrons only occasionally.
The r-process, in contrast, can produce the entire range of heavy elements in one spectacular flash of creation that barely lasts a second. In this scenario, neutrons are plentiful and slam into nuclei one after another before they have time to decay. A nucleus can rapidly balloon into a highly unstable isotope, going all the way up to what’s called the neutron drip line—the absolute limit of the neutron-to-proton ratio allowed by nature inside a nucleus. The extremely heavy nucleus will then convert many of its neutrons to protons via beta decays or even break into smaller nuclei, ultimately producing a range of stable heavy elements. Many details about how this plays out are unclear. After a nucleus absorbs extra neutrons, for instance, but before it becomes stable, exotic nuclei arise that scientists don’t understand. These in-between nuclei have properties that push the bounds of physics, and measuring them in a laboratory is difficult and sometimes even impossible.
Over the years scientists proposed many places in the universe where the r-process might occur, but the truth remained a mystery—among the greatest in nuclear astrophysics—for more than six decades. For a long time they thought core-collapse supernovae—explosive deaths of stars more than eight to 10 times the mass of our sun—might host the r-process. But simulations of typical core-collapse supernovae couldn’t reproduce the neutron richness and thermodynamic conditions needed except, perhaps, in the case of rare explosions driven by strong magnetic fields. In 1974 James M. Lattimer and David N. Schramm suggested that decompressing neutron star matter could provide the ingredients for the r-process.
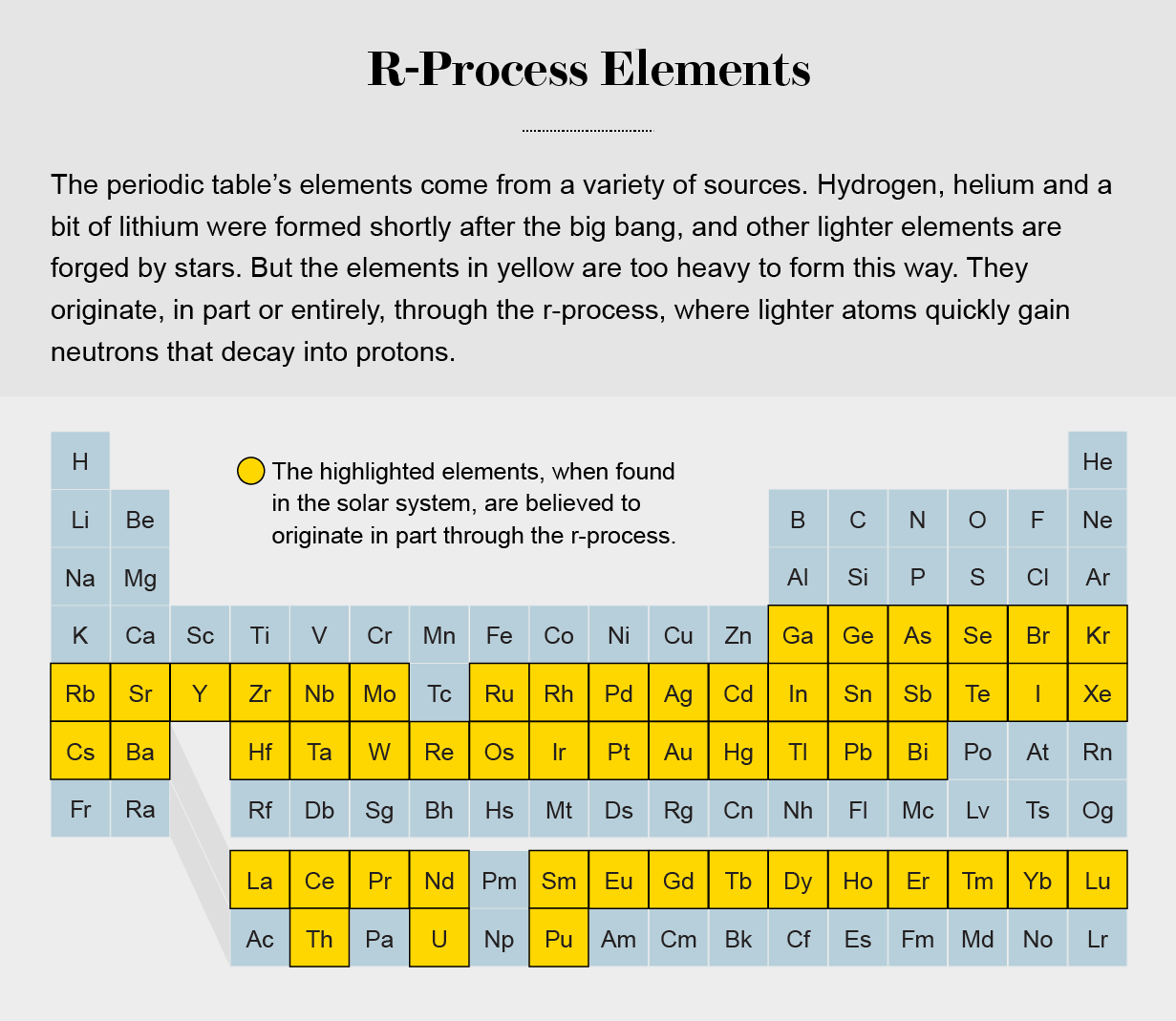
A neutron star is born when a massive star runs out of nuclear fuel and its gravity causes the core to collapse inward. The overwhelming force of the star’s mass on the core compresses it to extremely high densities, causing electrons and protons to fuse together to become neutrons. While the rest of the star gets expelled in the supernova, the neutron star remains intact—a compact remnant containing the densest matter known in the universe. Neutron stars more massive than a certain limit further collapse into black holes, but we don’t know the exact point of this transition, nor do we know how “squishy” they are. The inner structure of neutron stars is an open question. They might contain mostly neutrons and a small fraction of protons inside a crust of heavier nuclei at their surfaces. But their interiors could be even weirder than that. Deep inside the neutron star, matter may take on truly bizarre forms, ranging from a soup of quarks and gluons—the particles that make up normal matter—to a sea of “hyperons,” which are made of so-called strange quarks.
Lattimer and Schramm proposed that neutron-rich matter is ejected when a neutron star collides with a black hole. But by 1982 scientists favored a scenario involving two neutron stars smashing together. While some researchers were working to understand how these crashes could synthesize new elements, others were trying to predict what kind of light we would expect to see from a neutron star merger. Some people suggested a connection between neutron star collisions and gamma-ray bursts—highly energetic explosions in space that emit a flash of gamma rays. And because r-process nuclei would be unstable and undergo radioactive decay, they should be able to heat up the material surrounding them and power an electromagnetic flare that would carry signatures of the elements produced. In 2010 Brian Metzger and his collaborators introduced the term “kilonova” to refer to such flares (first proposed in 1998) after determining that they would be approximately 1,000 times brighter than a regular flash of light called a nova.
Despite this intense theoretical development, there was little direct confirmation until just a few years ago, when one remarkable set of observations saw straight into the heart of a neutron star merger.
A Cosmic Symphony
In 2015 the Laser Interferometer Gravitational-wave Observatory (LIGO) did something extraordinary: it made the first observation of gravitational waves, which were generated by two black holes spiraling toward each other and merging. The detection was designated GW150914. At the time I was a graduate student at North Carolina State University. I remember watching the announcement along with the entire physics department in the common area of our building, feeling deeply moved. I tried to absorb everything I could about this new window to our universe. I learned that neutron star mergers produce less energy than black hole mergers, so they are more difficult to detect. But I and other scientists held out hope that soon the experiment would find them as well.
A couple of years passed, and LIGO and its sibling observatory Virgo detected more binary black hole collisions. Yet neutron star mergers remained elusive. Then, in the fall of 2017, I heard rumors that LIGO-Virgo had seen a neutron star collision for the first time. The rumors hinted that in addition to the gravitational-wave signal, astronomers had observed a short gamma-ray burst and something that looked a lot like a kilonova. The excitement among physicists was intense.
Soon enough, I was watching scientists from LIGO and various telescopes around the world announce the gravitational-wave observation, called GW170817, and the associated electromagnetic signals. I was awed by the amount of new knowledge these observations had already generated. The very next day there were almost 70 new papers about GW170817 on arXiv.org, a website where researchers can publish early, unreviewed versions of their papers. The event forecasted the promise of multimessenger astronomy—the ability to see cosmic phenomena through different “messengers” and combine the information to achieve a fuller understanding of the event. This was the first time astronomers saw gravitational waves and light—including radio, optical, x-ray and gamma-ray light—coming from the same celestial source.
The gravitational waves seen by LIGO-Virgo originated in the crash of a pair of neutron stars about 130 million light-years from Earth. This may seem far, but it’s actually close for a gravitational-wave source. The details of the signal, such as how the waves’ frequency and strength changed with time, allowed researchers to estimate that each neutron star had weighed about 1.17 to 1.6 times the mass of our sun and had a radius of roughly 11 to 12 kilometers.
As soon as the gravitational-wave signal arrived, astronomers followed up with conventional telescopes. Working together, LIGO and Virgo narrowed the location range for GW170817 to a much smaller region of the sky than in previous gravitational-wave events. Roughly 1.7 seconds after the gravitational waves came in, gamma-ray telescopes Fermi-GBM and INTEGRAL detected a faint burst of gamma rays lasting only a couple of seconds that came from the same direction as GW170817. This discovery definitively linked neutron star mergers with short gamma-ray bursts for the first time. But there was more! Images taken with the Henrietta Swopes one-meter telescope at the Las Campanas Observatory in Chile showed a new source of light located in the old but bright galaxy NGC 4993. By breaking up the light into its constituent colors and examining its spectrum, astronomers concluded that the signal was consistent with the idea that heavy elements were being forged there. We were looking at a true kilonova.
The way the kilonova’s spectrum changed over time was interesting. Shorter wavelengths of light, which are bluer, peaked early, and longer, red wavelengths became predominant later. These peaks can be explained by the composition and velocity of the material ejected from the merger. A blue kilonova can be produced by fast-moving ejecta made mainly of lighter heavy elements without any “lanthanides”—the metallic periodic elements from lanthanum to lutetium, which are highly opaque to blue light. A red kilonova, in contrast, requires slow-moving ejecta containing lots of heavy elements, including lanthanides.
How does the merger generate these distinct components? This question puts us in uncertain territory, the realm of theory and simulations. Researchers are still trying to understand how the collision ejects material, what the material is made of and how the resulting kilonova unfolds. Kilonova spectra are very difficult to disentangle. Because the material is moving so fast, the fingerprints of various elements get smeared and blended together. We also lack reliable atomic data for many of the heavier elements, so it’s hard to predict what their spectral signatures look like. The only plausible detection of an individual element in the GW170817 kilonova spectrum so far is of strontium. This is enough, though, to show that the r-process took place.
The discovery of this singular event has confirmed decades of theoretical predictions. Astrophysicists have finally established a connection between neutron star mergers and short gamma-ray bursts. The kilonova spectrum carries signatures of heavy elements, confirming that neutron star mergers are at least one site where r-process elements are produced.
But a lot remains to be understood and discovered. The mechanism that produces short gamma-ray bursts in mergers is still unclear. Properties of matter ejected in a merger are also changed in important ways by neutrinos. Careful tracking of these particles and their interactions in theoretical models is necessary but challenging and often requires a prohibitively large amount of computational power. We also don’t know what object was created when the neutron stars merged. It could have been another neutron star, a neutron star on its way to becoming a black hole, or a black hole. Finally, although we now know that neutron star mergers can host the r-process, they are not the only places where it happens.
Observations of very old stars containing r-process elements suggest other possibilities, which include rare supernovae and collisions of neutron stars with black holes. We will not be able to uncover the origin of heavy elements with any one observation, no matter how extraordinary. GW170817 is just the beginning.
New Opportunities
We can’t expect all kilonovae to look the same as the one associated with GW170817. We suspect they come in many forms, each with distinctive features, and we’re in for a lot of surprises. In fact, astronomers at Northwestern University recently discovered a kilonova along with a long gamma-ray burst—an interesting combination suggesting that mergers can spawn gamma-ray bursts with longer light curves, too.
To understand the r-process, experts in several disciplines will have to work together: observational astronomers studying stars both old and new, gravitational-wave astronomers measuring distortions in spacetime, nuclear theorists constructing models of nuclear structures and of the matter inside neutron stars, experimental nuclear physicists tracking down the properties of unstable neutron-rich nuclei, and computational astrophysicists simulating events such as neutron star mergers by solving equations that take months to process on some of the largest computers in the world.
As existing gravitational-wave observatories become increasingly sensitive, new telescopes will come online to collect light from the transient sky. New projects such as the Facility for Rare Isotope Beams, which opened in May 2022 at Michigan State University, will measure the nuclear properties of rare nuclei. Proposed gravitational-wave observatories such as the ground-based Einstein Telescope are currently being planned in Europe.
Decades of progress in many fields have brought us to a point where we can investigate the origin of heavy elements in ways that were inaccessible just a few years ago. We are finally poised to put all the pieces together. Every isotope of every element in the periodic table has the potential to tell us something about the nuclear history of the universe.