The prairie vole is a small Midwestern rodent known for shacking up and settling down, a tendency that is rare among mammals. Mated pairs form bonds, share a nest and raise young together. In the laboratory, a pair-bonded vole will work for access to its mate. Prairie voles even exhibit something like empathy for their partners, getting stressed when they are stressed, and consoling each other through touch. As the pandemic has brought into stark relief, such social connections are essential to human well-being as well. Researchers are turning to these unusual rodents to understand how relationships have a profound impact on health.
Leveraging biomedical advances of the past few decades, scientists have watched neurons in action. They have manipulated gene activity with exquisite precision, examining the functions of individual genes in specific brain regions. With the prairie vole as a subject, researchers are learning how bonds are forged, how early life shapes relationships and why we ache when they fall apart.
Of course, prairie voles are not humans. And so these insights raise a question. How has a shaggy little rodent slightly smaller than a tennis ball and routinely mistaken for a mole, a mouse or a rat become a stunt double for the thrills of love and the perils of loss? The answer tells us as much about how science advances as it does about our own hearts.
Early Clues
Over the past two million years huge sheets of ice ground the landscape of central Illinois to a whetstone flatness. Today cornfields stretch to the horizon, but crowded into their interstices are fragments of the prairie that once covered this part of the state. One autumn nearly 50 years ago Lowell Getz, then a young ecologist at the University of Illinois, checked traps hidden in the grass and clover. One of the rodent species he captured, the prairie vole, behaved differently from the others, he noticed. Specific pairs of males and females kept showing up in traps together. In the 1970s zoologist Devra Kleiman had estimated that only about 3 percent of mammal species are monogamous. The data Getz and his students gathered suggested the prairie vole was among them.
Getz was not the first to suggest that prairie voles were monogamous, but his work attracted the attention of a colleague, behavioral endocrinologist Sue Carter, and together their research teams began to document the full range of vole social behaviors and the hormones that underpin them both in the lab and in the wild. Through studies carried out in the 1980s and 1990s, they found that males and females share a nest, raise young and defend a territory together. Carter’s lab developed a simple behavioral test to assess a vole’s “partner preference” that involved tethering a mate in one small chamber and an unfamiliar vole in another and then allowing a vole to choose between a partner and a stranger. Bonded prairie voles prefer to cuddle with a mate. Their bonds, which can last a lifetime, emerge after scandalously extensive mating.
Getz attributed the evolution of prairie vole bonding to the sparse distribution of food resources in their uniformly flat and grassy environment, which led to the wide scattering of voles across the landscape. Under such conditions, males were unable to reliably pursue multiple females, as other rodent species do, so it made more sense to settle down with one partner and defend a shared home. Females gained a partner to help with parental care and stave off intruders. Carter’s group found that the hormone oxytocin, long known as a regulator of birth, lactation and maternal care, was essential to forming bonds. A related hormone, vasopressin, soon emerged as another crucial regulator of prairie vole bonding.
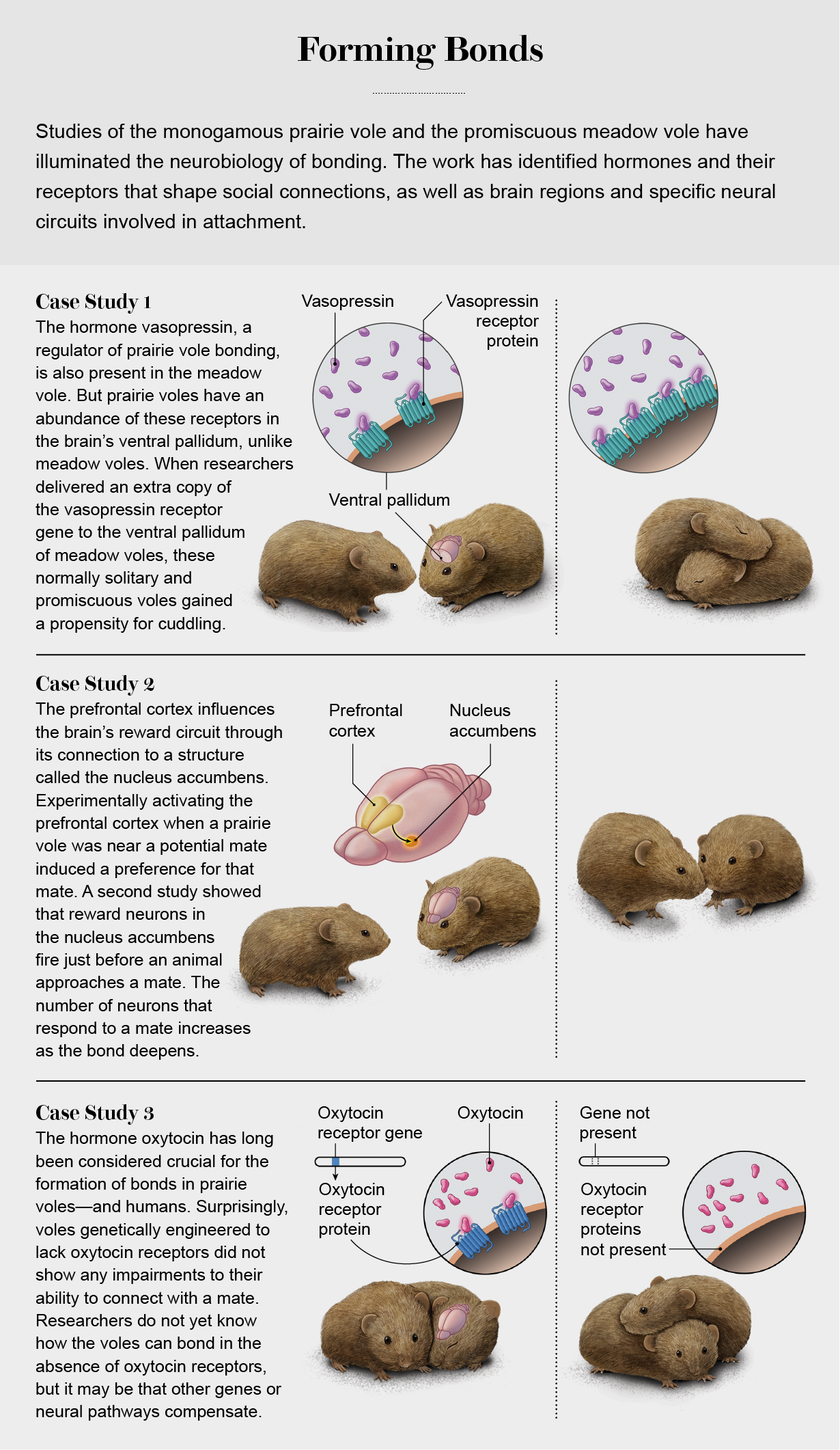
Oxytocin, vasopressin, and other closely related hormones are ubiquitous in nature. They have been found in nearly every animal species examined. But if these hormones occur in such a wide range of species, many of which are not monogamous, then their presence alone is surely not enough to make a species form pair-bonds. So how and why do these hormones shape bonding?
The answer lies in the way that hormones bring about changes in the brain. Hormones are small chemical compounds; in the case of vasopressin and oxytocin, they are small proteins known as peptides. Hormones influence the functions of the body’s cells by binding to large proteins known as receptors, whose shape and electrical charge interact with one particular hormone. When a hormone binds to its receptor protein, it causes a change in the receptor’s shape that triggers changes within the cell.
Because oxytocin and vasopressin are present in many species but promote bonding only in some species, it seemed plausible that there might be species differences in the distribution of hormone receptors. In the 1990s Tom Insel of the National Institutes of Health and his colleagues discovered that prairie voles and their monogamous relatives, pine voles, have receptors for oxytocin and vasopressin in different brain regions than their promiscuous relatives, the meadow and montane voles. Whereas the monogamous voles have abundant receptors for these hormones in the nucleus accumbens and the ventral pallidum, structures that are part of the brain’s reward circuit, promiscuous voles largely lack receptors in these brain areas. These are the same regions neuroscientists have long studied in the context of drug abuse. Headlines soon announced that love was addictive.
The findings supported the idea that differences in the distribution of hormone receptors could account for the different behaviors of promiscuous and monogamous voles. But to understand exactly how hormone receptors shape bonding, researchers needed to manipulate the genes that encode the receptors. The tools for doing that kind of work would come from an unexpected source.
New Tools, New Revelations
In the late 1960s, even before ecologists were starting to wonder about the social lives of prairie voles, virologists made a discovery that would eventually lead to the development of a novel tool for studying genes, brains and behavior. The scientists were examining the DNA of a group of viruses called adenoviruses, which cause the common cold. They found that their adenovirus samples were contaminated by viruslike particles that they called adeno-associated viruses (AAV). Whereas viruses need a host cell to reproduce, an AAV needs both a cell and the co-infection of an adenovirus to multiply. It’s a parasite’s parasite. If an AAV infects a human cell that lacks an adenovirus, it simply lies in wait until one comes along.
The fact that an AAV can enter and live peaceably inside a cell makes it an excellent vector for delivering DNA to change a cell’s workings. In the 1990s researchers began engineering AAV to tweak the neurons of rats and mice in an effort to figure out what they do. The tools they developed, we soon learned, worked just as well on voles. To study the role of hormones in pair-bonds, Larry Young of Emory University and his colleagues used AAV to deliver an extra copy of the vasopressin receptor gene into the ventral pallidum of meadow voles. With their vasopressin receptor levels boosted in this brain region, these normally solitary and promiscuous voles gained a new propensity to cuddle with a mate. The work showed that the abundance of vasopressin receptors in the brain’s reward circuits explained at least some of the behavioral differences between monogamous and promiscuous voles.
AAV has also allowed researchers to actually watch bond formation in real time. When scientists engineered a novel light-activated protein that could alter the electrical activity of a neuron, neurobiologists used an AAV to put this protein into the prefrontal cortex, a brain region that influences reward through its contact with the nucleus accumbens. Elizabeth Amadei, Robert Liu and their colleagues at Emory University showed that activating these neurons when a vole was near a potential partner was enough to generate a preference for the would-be mate. Another group, led by one of us (Donaldson), used AAV to introduce a protein that glows when a neuron is active into the brains of voles. Using tiny head-mounted microscopes, the researchers could see what was happening in the brain as voles formed a bond. They found that reward neurons in the nucleus accumbens light up just before an animal approaches a mate. Remarkably, the number of neurons that respond to a mate also increases as a bond deepens over time.
The advent of CRISPR DNA-editing technology a decade ago has given investigators new and unprecedented control over genes and the work they do. CRISPR, an acronym for clustered regularly interspaced short palindromic repeats, operates like a molecular scalpel to make incisions in DNA. Tailoring genomes with CRISPR is complex and costly, but the technology has also upended our understanding of oxytocin, the so-called love hormone.
Decades of research implicates oxytocin in the formation of prairie vole pair-bonds. And several studies suggest that oxytocin acts in reward circuits to shape human bonds as well. It seemed a promising experiment, then, when one of us (Manoli) teamed up with colleagues to use CRISPR to delete the gene that encodes the oxytocin receptor in prairie vole embryos. We expected the genetically modified voles to exhibit impairments in their ability to bond with mates. But shockingly, prairie voles that lacked the oxytocin receptor altogether actually formed preferences for mates as readily as their genetically unmanipulated siblings.
How can this be? Honestly, we don’t yet know. One idea is that during development other genes or neural pathways naturally compensate for the lack of oxytocin receptors. We already know that there are many other genes that influence pair-bonding, not only oxytocin, vasopressin and their receptors. The use of CRISPR has revealed that a piece of music we imagined had been written for a small group is really a symphony. Transcribing this new, more complex music will deepen our understanding of attachment and its underlying mechanisms.
Beyond Oxytocin and Vasopressin
The discovery that the oxytocin receptor is not strictly necessary for prairie vole bonding demonstrates that however important the genes encoding oxytocin, vasopressin and their receptors may be, they are not the whole story. Other 21st-century tools are helping scientists fill in the gaps in our understanding of how social connections form—and how they rewire the brain.
The past decade of work in gene sequencing has made it possible to exhaustively quantify the genes that are active in any particular brain region. This genome-wide approach to looking for genes and other DNA sequences associated with certain behaviors has its own challenges, but it offers a view that is “unbiased” in the sense that it looks beyond the small set of players scientists already think are important for bonding.
One such study examined gene activity across brain regions during bond formation. It found that most differences between the monogamous prairie vole and the promiscuous meadow vole were evident even before bonding began, as though their brains were already prepared for their specific social behaviors. After the voles had mated repeatedly, a subset of genes turned on that are particularly important for learning and memory—the kind of rewiring one might expect to happen as an animal transitions from being single to being paired with a specific partner. Another study found that distinctive genes are turned on in the brain’s reward structures as the bond becomes stable. These changes reverse if the bond is broken by prolonged separation.
Just as new genome sequencing methods have offered fresh perspectives on DNA and its function, parallel advances in the microscopic study of biological tissues have expanded our view of the brain. Traditionally, studying the microanatomy of tissue has required that investigators obtain a thin slice of tissue for examination. We can now render a tissue transparent, allowing researchers to image an entire brain without the need to physically slice it. Like genome-wide studies, this approach to studying the brain offers an unbiased view. By examining cleared brains for a protein that is produced in response to neural activity, one of us (Phelps), along with Pavel Osten, then at Cold Spring Harbor Laboratory, and other colleagues, made the first brain-wide map of regions that are active as prairie voles turn mating into bonds. The results confirm earlier work suggesting that reward circuits are involved in bonding but also implicate many other brain regions. They show that in both males and females, neural activity follows a path known to be important in sexual responses. This neural pathway finds its way into nearly 70 distinct brain regions, eliciting a storm of activity as brains rewire themselves for a bond. And just as Sue Carter suggested decades ago, sex itself seems to drive this rewiring.
Once a bond has developed, the neural activity is concentrated in a much smaller circuit. Connections between the amygdala and hypothalamus, brain regions essential to both emotional learning and hormone release, come alive. These same connections have recently been shown to shape nonsexual social connections in lab mice, and the new results suggest some common mechanisms of social attachments across both species and categories of bonds. Together these unbiased approaches promise a complete catalog of the genes and brain regions that enable a bond to form and persist or that allow it to dissolve in time.
From Voles to Humans
In the middle of the 20th century British psychologist John Bowlby and American-Canadian psychologist Mary Ainsworth drew on the work of animal behaviorists to suggest that a child’s need for love was fundamental to human biology. Bowlby posited that our attachments represented a specialized evolved neural system—that is, an evolutionarily adaptive brain mechanism that helped us successfully navigate childhood by binding us to our caregivers. Although Bowlby and Ainsworth’s attachment theory was considered radical in its time, scientists have since expanded it to explain not only human parenting but also friendships, romantic relationships and the pang of their loss.
Prairie vole bonds and the mechanisms that underlie their formation and influence provide a concrete example of what such an evolved neural system might look like. We see that bonds rely on joining the specific cues associated with a potential partner to the feelings of desire. Reward prompts voles to stay close to each other, to huddle together. There are genes that stand ready to guide circuits toward learning the identity of a new partner, genes that seem to stabilize bonds, and genes that oversee the experience of loss. To do so, they must, in ways we do not yet fully understand, harness the brain’s capacity for memory and emotion.
This is not to say that the vole’s experience of pair-bonding is exactly like the human experience of love. Neuropsychologists have built on the framework derived from prairie vole research to suggest that centers of emotion and reward interact with other brain regions—areas that promote empathy and perspective taking, for example—to produce the rich sense of what it means to be in love. The view implies that romantic love has an emotional core similar to that experienced by other animals but enriched by our complex understanding of ourselves and our most significant others.
Human studies inspired by prairie vole findings support the comparison of the two species. Love is so essential to the human experience that scientists long assumed its biological basis must reside in our cerebral cortex, the brain’s presumed center of thought. This part of the brain expanded considerably during primate evolution, which suggests that it has played an important role in the success of our branch of the mammal family tree. Work on prairie voles, however, inspired neuropsychologists to look at more ancient structures, in the same reward regions implicated in prairie vole bonding. In one study, scientists asked people in relationships to rate just how in love they were with a partner. They found that these ratings predicted how much blood flowed to their reward systems when viewing pictures of their partners. Likewise, when a human subject holds her partner’s hand, it activates her nucleus accumbens—one of the brain regions that in prairie voles has receptors for oxytocin and vasopressin.
Our understanding of the hormonal regulators of human affections also seems consistent with our understanding of prairie vole pair-bonding. Humans get a flush of oxytocin in response to a tender caress or to orgasm. But it’s a versatile hormone: it also surges when we make eye contact with a large-eyed puppy.
Scientists hope to one day grasp human bonding well enough to be able to intervene when it causes pain—to lessen, for example, the ravages of chronic loneliness or to dull the edges of a devastating grief. Drugs intended to mimic the functions of oxytocin and vasopressin have, so far, not lived up to their therapeutic potential. And as we have seen, even among prairie voles the mechanisms of bonding are not entirely understood.
To fully comprehend bonds and their consequences, we need science rich enough to accommodate ecology, evolution, neuroscience and molecular genetics, each of which offers a complementary view on how and why bonds form. It requires basic research. The same technological advances that have made the prairie vole such an exciting animal in which to study attachment are opening up new avenues of study in other species, such as the parental care of poison frogs and the conversations of fruit bats. The knowledge generated from such studies is valuable for its own sake, and what we discover may one day transform our lives. New species and new tools mean new perspectives on life—and love.